Cell Mechanics and Modeling
The transmission of physiologic (mechanical) loads requires participation of multiple components of a joint, including bone, muscles, tendons/ligaments and cartilage. Because the function of cartilage is to support time-dependent loading, the response of the tissue and its constituents are extremely important. Not only does cartilage compression causes matrix deformation, but it also deforms the cells within the tissue (Video 1.; Figs. 1 & 2). The mechanical deformation of cells within the tissue stimulates cellular metabolism [1, 2] which in turn, regulates the tissue’s environment.
It is this conversion of mechanical signals (from compression) to chemical signals (see [3]) within the cell that modulate biochemical activity (e.g. protein synthesis see [4-6]) and changes in chondrocyte gene expression (see [7]) and is referred to as mechanotransduction. Cell-matrix interactions are essential for maintaining the integrity of AC and an intact matrix is essential for the transmission of mechanical signals and hence, cell survival. Because abnormal loading or mechanical injuries to AC are known to shift the balance in catabolic activity over anabolic processes in chondrocytes [1, 2], these can produce degradative processes that may eventually lead to the development of OA.
Research in the field of chondrocyte biomechanics involves understanding how these cells respond to loading, through mechanical deformation. Manipulation of cartilage enzymatically, chemically or through the utilization of surgical interventions (in whole joints) is being performed in order to study how cells respond to mechanical deformation. This research is important in characterizing the tissue’s macro-level tissue properties and/or whole joint-level responses during load bearing.
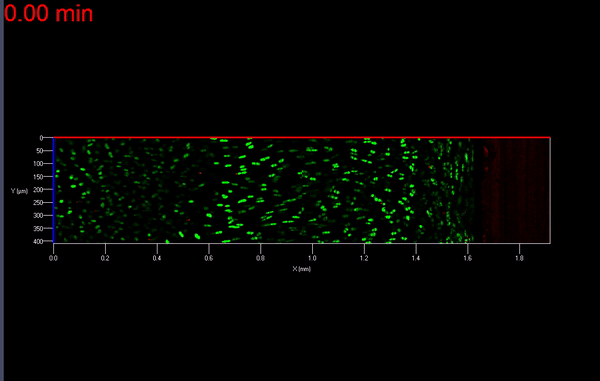
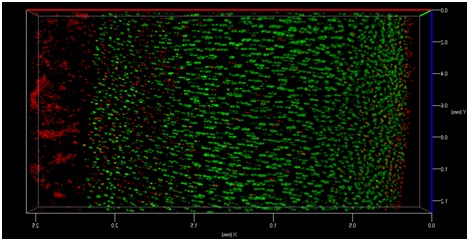
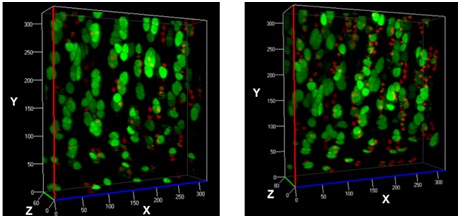
As mentioned earlier, the damage to the extracellular matrix of cartilage may alter enzymatic pathways of the chondrocyte. Yet, direct observations of these changes are very difficult if not impossible to obtain. However, it is possible to address the effect of mechanical signals between cell-matrix structures with the help of biomechanical modeling. For instance, the aforementioned macro scale loading conditions can be used as input parameters for cell scale biomechanical model.
It is known that the properties of cartilage have a great influence on cell deformations in mechanically loaded cartilage [8-11]. However, limited information exists about the role of different components (collagen, proteoglycans, fluid) in cartilage, and in close proximity to chondrocytes, how these can modulate cell deformation within the tissue.
By implementing experimentally detected cartilage composition data and combining it with experimental loading conditions, we can estimate the effect of these factors in our biomechanical model. Thus, we may address mechanisms for the changes in cell morphology and cartilage at different stages of osteoarthritis. Ultimately, biomechanical models may allow us to find a way to prevent these undesirable changes in the cell morphology, enabling us to restore the cell and tissue functionality.
References
- Ramage L, Nuki G, Salter DM (2009), Signalling cascades in mechanotransduction: cell–matrix interactions and mechanical loading. Scandanvian Journal of Medicine and Science in Sports, 19, 457–469.
- Leong DJ, Hardin JA, Cobelli NJ, Sun HB (2011), Mechanotransduction and cartilage integrity. Annals of the New York Academy of Sciences, 1240, 32-37.
- Han S-K, Wouters W, Clark A, Herzog W (2012), Mechanically induced calcium signaling in chondrocytes in situ. Journal of Orthopaedic Research, 30, 475-481.
- Wong M, Wuethrich P, Eggli P Hunziker E (1996), Zone-specific cell biosynthetic activity in mature bovine articular cartilage: a new method using confocal microscopic stereology and quantitative autoradiography. Journal of Orthopaedic Research, 14, 424-432.
- Parkkinen JJ, Lammi MJ, Helminen HJ, Tammi M (1992), Local stimulation of proteoglycan synthesis in articular cartilage explants by dynamic compression in vitro. Journal of Orthopaedic Research, 10, 610-620.
- Moskowitz RW, Goldberg VM, Malemud CJ (1981), Metabolic responses of cartilage in experimentally induced osteoarthritis. Annals of the Rheumatic Diseases, 40, 584-592.
- Bevill S, Briant P, Levenston M, Andiacchi T (2009), Central and peripheral region tibial plateau chondrocytes respond differently to in vitro dynamic compression. Osteoarthritic and Cartilage, 17, 980-987.
- Quinn, T.M., et al. (2001), Static compression of articular cartilage can reduce solute diffusivity and partitioning: implications for the chondrocyte biological response. J Biomech, 34(11), 1463-9.
- Quinn, T.M., et al. (1999), Physical and biological regulation of proteoglycan turnover around chondrocytes in cartilage explants. Implications for tissue degradation and repair. Annals of the New York Academy of Sciences, 878, 420-41.
- Han, S.K., et al. (2010), Mechanical loading of in situ chondrocytes in lapine retropatellar cartilage after anterior cruciate ligament transection. Journal of the Royal Society Interface, 7(47), 895-903.
- Tanska, P et al. (2013), Superficial collagen fibril modulus and pericellular fixed charge density modulate chondrocyte volumetric behaviour in early osteoarthritis. Computational and Mathematical Methods in Medicine, 2013, http://dx.doi.org/10.1155/2013/164146
Ph.D. theses of our researchers
Siru Turunen:
Osmotic and Mechanical Loading of Chondrocytes in Situ
Publications of our researchers
- Turunen SM, Lammi MJ, Saarakkala S, Han SK, Herzog W, Tanska P and Korhonen RK. The effect of collagen degradation on chondrocyte volume and morphology in bovine articular cartilage following a hypotonic challenge. Biomech Model Mechanobiol. 2013 Jun;12(3):417-29. doi: 10.1007/s10237-012-0409-4.
- Turunen SM, Han SK, Herzog W and Korhonen RK. Cell deformation behavior in mechanically loaded rabbit articular cartilage 4 weeks after anterior cruciate ligament transection. Osteoarthritis Cartilage. 2013 Mar;21(3):505-13. doi: 10.1016/j.joca.2012.12.001.
- Julkunen P, Wilson W, Isaksson H, Jurvelin JS, Herzog W and Korhonen RK. A review of the combination of experimental measurements and fibril-reinforced modeling for investigation of articular cartilage and chondrocyte response to loading. Comput Math Methods Med. 2013;2013:326150. doi: 10.1155/2013/326150. Epub 2013
- Tanska P, Turunen SM, Han SK, Julkunen P, Herzog W and Korhonen RK. Superficial collagen fibril modulus and pericellular fixed charge density modulate chondrocyte volumetric behaviour in early osteoarthritis. Comput Math Methods Med. 2013;2013:164146. doi: 10.1155/2013/164146. Epub 2013
- Huttu M, Turunen S, Sokolinski V, Tiitu V, Lammi M and Korhonen RK. Effects of Medium and Temperature on Cellular Responses in the Superficial Zone of Hypo-Osmotically Challenged Articular Cartilage. J Funct Biomater. 2012 Sep;3(3):544-555.
- Turunen SM, Lammi MJ, Saarakkala S, Koistinen A and Korhonen RK. Hypotonic challenge modulates cell volumes differently in the superficial zone of intact articular cartilage and cartilage explant. Biomech Model Mechanobiol. 2012 May;11(5):665-75. doi: 10.1007/s10237-011-0341-z.
- Turunen MJ, Toyras J, Lammi MJ, Jurvelin JS and Korhonen RK. Hyperosmolaric contrast agents in cartilage tomography may expose cartilage to overload-induced cell death. J Biomech. 2012 Feb 2;45(3):497-503. doi: 10.1016/j.jbiomech.2011.11.049. Epub
- Korhonen RK, Han SK and Herzog W. Osmotic loading of in situ chondrocytes in their native environment. Mol Cell Biomech. 2010 Sep;7(3):125-34.
- Korhonen RK, Han SK and Herzog W. Osmotic loading of articular cartilage modulates cell deformations along primary collagen fibril directions. J Biomech. 2010 Mar 3;43(4):783-7. doi: 10.1016/j.jbiomech.2009.10.022. Epub 2009
- Julkunen P, Wilson W, Jurvelin JS and Korhonen RK. Composition of the pericellular matrix modulates the deformation behaviour of chondrocytes in articular cartilage under static loading. Med Biol Eng Comput. 2009 Dec;47(12):1281-90. doi: 10.1007/s11517-009-0547-8.
- Korhonen RK, Julkunen P, Wilson W and Herzog W. Importance of collagen orientation and depth-dependent fixed charge densities of cartilage on mechanical behavior of chondrocytes. J Biomech Eng. 2008 Apr;130(2):021003. doi: 10.1115/1.2898725.
- Korhonen RK and Herzog W. Depth-dependent analysis of the role of collagen fibrils, fixed charges and fluid in the pericellular matrix of articular cartilage on chondrocyte mechanics. J Biomech. 2008;41(2):480-5. Epub 2007 Oct 22.
- Korhonen RK, Julkunen P, Rieppo J, Lappalainen R, Konttinen YT and Jurvelin JS. Collagen network of articular cartilage modulates fluid flow and mechanical stresses in chondrocyte. Biomech Model Mechanobiol. 2006 Jun;5(2-3):150-9. Epub 2006 Feb 28.